Originally Published: on Oct. 1, 2017 | American Journal of Physiology | By James R. Broatch, Aaron Petersen, and David J. Bishop
Cold-water immersion following sprint interval training does not alter endurance signaling pathways or training adaptations in human skeletal muscle.
Broatch JR, Petersen A, Bishop DJ. Cold-water immersion following sprint interval training does not alter endurance signaling pathways or training adaptations in human skeletal muscle. Am J Physiol Regul Integr Comp Physiol 313: R372–R384, 2017. First published July 5, 2017; doi:10.1152/ajpregu.00434.2016.—We investigated the underlying molecular mechanisms by which postexercise cold-water immersion (CWI) may alter key markers of mitochondrial biogenesis following both a single session and 6 wk of sprint interval training (SIT). Nineteen men performed a single SIT session, followed by one of two 15-min recovery conditions: cold-water immersion (10°C) or a passive room temperature control (23°C). Sixteen of these participants also completed 6 wk of SIT, each session followed immediately by their designated recovery condition. Four muscle biopsies were obtained in total, three during the single SIT session (preexercise, postrecovery, and 3 h postrecovery) and one 48 h after the last SIT session. After a single SIT session, phosphorylated (p-)AMPK, p-p38 MAPK, p-p53, and peroxisome proliferator-activated receptor- coactivator-1 (PGC-1) mRNA were all increased (P 0.05). Postexercise CWI had no effect on these responses. Consistent with the lack of a response after a single session, regular postexercise CWI had no effect on PGC-1 or p53 protein content. Six weeks of SIT increased peak aerobic power, maximal oxygen consumption, maximal uncoupled respiration (complexes I and II), and 2-km time trial performance (P 0.05). However, regular CWI had no effect on changes in these markers, consistent with the lack of response in the markers of mitochondrial biogenesis. Although these observations suggest that CWI is not detrimental to endurance adaptations following 6 wk of SIT, they question whether postexercise CWI is an effective strategy to promote mitochondrial biogenesis and improvements in endurance performance. cold-shock proteins; cryotherapy; exercise recovery; tumor suppressor p53; citrate synthase DESPITE THE POPULARITY of postexercise cold-water immersion (CWI) in athletic training regimes, few studies have investigated its effect on the adaptive response to endurance-related training. Notably, there have been no studies investigating the use of CWI in conjunction with sprint interval training (SIT). Some research involving lower intensity exercise indicates that CWI may stimulate a number of cellular responses related to skeletal muscle mitochondrial biogenesis following a single exercise session in humans (1, 33, 34). Furthermore, improvements in skeletal muscle recovery following CWI (67) may allow athletes to train with a higher quality and/or load in subsequent sessions, potentially providing a greater stimulus for adaptation (29). However, these benefits may be counteracted by the attenuation of molecular responses related to vascular remodeling and heat shock protein induction (74), resulting in long-term detrimental effects on skeletal muscle adaptations and endurance performance. Given the contrasting findings reported to date, clarification of the effects of CWI on both molecular responses to a single exercise session and adaptations to exercise training are warranted. In humans, postexercise exposure to cold has been reported to augment increases in peroxisome proliferator-activated receptor- coactivator-1 (PGC-1) mRNA (34, 61, 62), an important regulator of skeletal muscle mitochondrial biogenesis (41). Increases in PGC-1 mRNA after postexercise muscle cooling have been attributed to both shivering and nonshivering thermogenesis (34, 61, 62). For example, 1 h of exercise followed by 3 h of recovery in a cold (7°C) environment induced significantly greater increases in PGC-1 mRNA in human skeletal muscle compared with a room temperature (20°C) control condition (62). The increase in PGC-1 mRNA reported by Slivka et al. (62) was attributed in part to contraction-induced increases in calcium (Ca2) and adenosine monophosphate (AMP) as a result of shivering. Apart from producing heat via repetitive muscle contraction and subsequent energy expenditure, shivering may also serve to activate PGC-1 via the upstream kinases calcium/calmodulin-dependent protein kinase and AMP-activated protein kinase (AMPK; 62). Consistent with work in adipose tissue and muscle cells (53, 72), PGC-1 mRNA has also been reported to increase in human skeletal muscle following postexercise CWI via nonshivering thermogenesis (34), a process by which heat is produced from shivering-independent mechanisms such as mitochondrial uncoupling and substrate cycling (70). This cold-induced increase in PGC-1 mRNA may be related to -adrenergic stimulation and nitric oxide synthesis (34) and/or altered calcium handling and subsequent p38 MAPK/AMPK phosphorylation (33). These studies suggest that cold application may be a novel method to promote exercise-induced mitochondrial biogenesis in humans, but further research is required to identify additional underlying mechanisms.
Another key transcription factor involved in the regulation of exercise-induced mitochondrial biogenesis is p53 (58). As shown in a number of different cell and tissue types, p53 plays an important role in regulating mitochondrial content, oxidative capacity, and exercise performance (44, 50, 56, 58, 59). Apart from its well-defined role in response to genotoxic stress and associated DNA damage, research has also implicated p53 activation in response to everyday stressors (e.g., metabolic stress from exercise; 9). Activation of p53 has also been reported following cold stress (28°C) in cultured cells (43), implicating the cold stress imposed by postexercise CWI as a potential stimulus to augment exercise-induced increases in p53 activation. Once activated, p53 transcriptionally regulates genes associated with energy metabolism and mitochondrial biogenesis (9, 28), including mitochondrial transcription factor (Tfam; 59), apoptosis-inducing factor (AIF; 64), mitofusin-2 (Mfn2; 68), dynamin-related protein-1 (Drp1; 39), and synthesis of cytochrome c oxidase 2 (SCO2; 44). Should cold stress increase p53 activity, this would provide an alternate explanation by which postexercise CWI might augment exerciseinduced mitochondrial biogenesis. Two studies have investigated the effects of regular postexercise CWI on skeletal muscle adaptations to high-intensity endurance training (1, 33). Consistent with a greater increase in PGC-1 mRNA after a single session of high-intensity running followed by CWI (34), regular postexercise CWI (administered during 4 wk of training) was reported to increase the abundance of a number of proteins associated with mitochondrial biogenesis [e.g., PGC-1, subunits of respiratory complexes I and III, and -hydroxyacyl-CoA dehydrogenase (-HAD); 33]. In contrast, a recent study by Aguiar et al. (1) reported that regular CWI administered during 4 wk of high-intensity cycle training had no additional effect on training-induced increases in PGC-1 protein content. However, although one study has observed a significant increase in p53 protein content following 4 wk of sprint interval training (SIT; 28), no study has investigated the effects of regular CWI on the content of this transcription factor. Another major gap in the literature is that no study has investigated the effect of regular postexercise CWI on markers of mitochondrial function (e.g., mass-specific mitochondrial respiration). We investigated, for the first time in the same experiment, the effects of postexercise CWI on mitochondrial content and function and some of the underlying molecular mechanisms. The present investigation was divided into two studies (a single exercise trial with biopsies and a training intervention). The aim of the biopsy trial was to investigate the response of the p53 pathway and other molecular mechanisms following a single exposure to postexercise CWI. The aim of the training intervention was to investigate the effects of regular postexercise CWI on the protein content of these transcription factors, as well as mitochondrial content and function. It was hypothesized that CWI administered after SIT would augment these molecular responses (independent of shivering, i.e., nonshivering thermogenesis), consistent with the upregulation of PGC-1 gene expression and protein accumulation reported when CWI was administered following high-intensity running (33, 34). It was also hypothesized that a CWIinduced increase in p53 signaling and protein accumulation would provide a basis for improved aerobic phenotype adaptations, including mitochondrial respiration, maximal oxygen consumption (V˙ O2peak), and endurance exercise performance. Considering the widespread use and acceptance by athletes of CWI as a recovery modality following training, the overall aim of this research was to provide mechanistic insights into its effects on skeletal muscle adaptations to training. METHODS Participants Recruited participants were recreationally active, performing some form of aerobic exercise (e.g., running or cycling) for ~30 – 60 min per day, ~2–3 days per week. Participants were not involved in a structured training regime or specifically trained to compete in any one sport. Written informed consent was obtained before participation, and all participants were screened for cardiovascular risk factors associated with exercise. All procedures were approved by the Institute of Sport, Exercise and Active Living Human Research Ethics Committee. The study followed a two-group parallel design, in which participants were assigned to one of two recovery conditions in a randomized, counterbalanced fashion. These conditions were a passive control (CON) or cold-water immersion (COLD). Briefly, the experimental protocol consisted of 1) familiarization and baseline testing, 2) a single exercise trial with biopsies, 3) a 6-wk SIT intervention, and 4) posttraining testing. Nineteen healthy men began the study and completed the biopsy trial (CON, n 10; COLD, n 9). Of these 19 participants, 16 completed the 6-wk training intervention and all posttraining measures (CON, n 8; COLD, n 8). Participant characteristics are detailed in Table 1. Familiarization Before baseline testing, participants made several familiarization visits to the laboratory to become accustomed with the equipment and testing procedures. These sessions included a graded exercise test (GXT), a 2-km cycling time trial (TT2), and 20-km cycling time trial (TT20), all performed on isolated days and separated by at least 24 h. Participants also completed a familiarization session of the SIT and their assigned recovery condition. Baseline Testing Participants completed baseline trials of the TT2, TT20, and GXT protocols. All baseline trials were separated by at least 24 h and performed in the morning (0700 –1000) after an overnight fast. Participants were asked to refrain from exercise and alcohol consumption for 24 h before each trial. Biopsy Trial After an overnight fast and abstinence from exercise and alcohol for the preceding 24 h, participants reported to the laboratory in the morning (0730) for the biopsy trial. Upon arrival, the lateral aspect of Table 1.
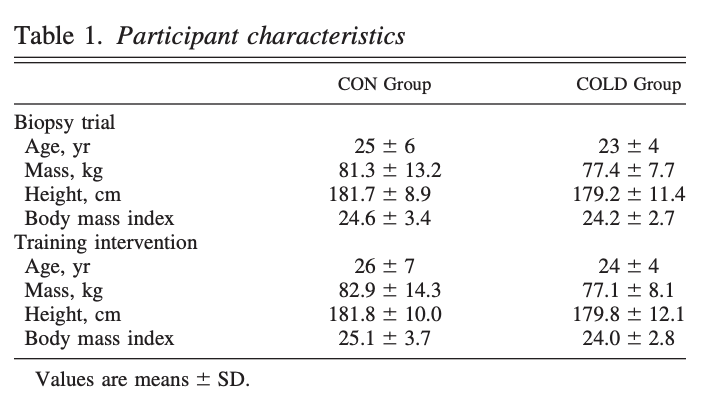
Familiarization Before baseline testing, participants made several familiarization visits to the laboratory to become accustomed with the equipment and testing procedures. These sessions included a graded exercise test (GXT), a 2-km cycling time trial (TT2), and 20-km cycling time trial (TT20), all performed on isolated days and separated by at least 24 h. Participants also completed a familiarization session of the SIT and their assigned recovery condition. Baseline Testing Participants completed baseline trials of the TT2, TT20, and GXT protocols. All baseline trials were separated by at least 24 h and performed in the morning (0700 –1000) after an overnight fast. Participants were asked to refrain from exercise and alcohol consumption for 24 h before each trial. Biopsy Trial After an overnight fast and abstinence from exercise and alcohol for the preceding 24 h, participants reported to the laboratory in the morning (0730) for the biopsy trial. Upon arrival, the lateral aspect of the right thigh (dominant leg for all participants) was anesthetized (1% Xylocaine). Three separate incisions were made through the skin and underlying fascia, corresponding to the three muscle biopsies taken during the experiment (preexercise, postrecovery, and 3 h postrecovery). The incisions were made approximately one-third of the distance from the knee to the hip, parallel to the long axis of the leg and spaced 1–2 cm apart. With the use of a 5-mm Bergström needle modified with suction (21), muscle biopsies were taken from the belly of the vastus lateralis. The first biopsy was taken at rest, immediately before the SIT session (Pre). Participants then performed the single SIT session and their designated recovery condition. Exactly 2 min postrecovery, a second muscle biopsy was taken (PostRec). Participants remained in the laboratory, fasted and at rest, until a third biopsy was taken 3 h postrecovery (3-h). The Post-Rec and 3-h biopsy incision sites were covered with sterile gauze and a waterproof dressing (Tegaderm; 3M, North Ryde, NSW, Australia) during exercise and recovery. After each biopsy, the corresponding incision site was closed with a suture. Samples were blotted on filter paper to remove blood and immediately snap frozen in liquid nitrogen and then stored at 80°C until subsequent analyses. Before being frozen, a small piece (~15–20 mg) of fresh muscle was sectioned from the pretraining biopsy for immediate analysis (within 5 min) of mitochondrial respiration. Participants were allowed to consume water ad libitum during the trial. This session doubled as the first training session for the 16 participants who subsequently completed the SIT intervention. Sprint Interval Training Intervention Sixteen participants completed three training sessions per week for 6 wk. On an electronically braked cycle ergometer (Velotron; RacerMate, Seattle, WA), participants first completed a 5-min warm-up at 75 W. This was immediately followed by 4 – 6 30-s “all-out” efforts at a constant resistance corresponding to 7.5–9.5% of body mass, separated by 4 min of rest. To ensure a progressive training stimulus during the 6-wk training period, participants performed four repetitions in weeks 1–2, five in weeks 3–4, and six in weeks 5–6. Resistance ranged from 7.5 to 9.5% of body mass, so that the fatigue-induced reduction in power during the 30-s bout was at least 20 W/s. To eliminate individual variance with self-administered “speeding up” of the flywheel, participants began each effort from a rolling start corresponding to 120 rpm. During each effort, participants were given extensive verbal encouragement and asked to remain seated in the saddle. This protocol has been shown to rapidly upregulate the oxidative phenotype, comparable to long-duration continuous endurance exercise (22, 23). Participants were asked to refrain from all other types of exercise during the training intervention. Posttraining Testing A resting muscle biopsy was taken ~48 –72 h after the final training session from the same leg used during the baseline testing (Post). Participants also completed posttraining trials for the TT2, TT20, and GXT protocols. The timing and nature of posttraining testing were identical to baseline testing. Exercise Performance Tests Time trials. On the same electronically braked cycle ergometer used during training, participants were instructed to complete TT2 and TT20 self-paced time trials as quickly as possible. The only feedback given to participants during the trials was an update of distance covered: the halfway mark (1 km) during the TT2 and every 2 km during the TT20. Participants were allowed to control the gear ratio throughout the entire time trial, corresponding to 3 gearings on the chain ring (28, 39, 52) and 10 on the rear sprocket (11, 17, 19, 21, 23). Heart rate (RS800sd; Polar Electro Oy, Kempele, Finland), exercise duration, and average power were recorded during the trial.
Graded exercise test. Participants performed a discontinuous GXT on an electronically braked cycle ergometer (Lode, Groningen, The Netherlands) to determine their lactate threshold (LT) and peak aerobic power (Wpeak). An intermittent protocol was used, with 4-min exercise stages and 30-s rest stages. After a 5-min steady-state warm-up at 75 W, the workload was increased by 30 W every 4.5 min until the participant reached volitional fatigue. The starting workload varied between 60 and 120 W, ascertained from the familiarization GXT, capping the number of stages to a maximum of 10. Participants were instructed to maintain a pedaling cadence of 70 rpm and wore a heart rate monitor during the test. The test was stopped when pedaling cadence dropped below 60 rpm. Blood samples for the GXT were taken from a venous catheter (Optiva IV Catheter 20-gauge 1.75; Smiths Medical) inserted into an antecubital vein 10 min before the first blood draw. The LT was determined as the workload at which venous blood lactate increased 1 mM above baseline (10) and was calculated using Lactate-E version 2.0 software (47). Wpeak was calculated as previously reported (30): Wpeak Wfinal t 240 · 30 where Wfinal is the power output of the last completed stage and t is the time in seconds of any final uncompleted stage. Peak oxygen uptake. After volitional fatigue in the GXT, participants performed 5 min of passive rest before performing a V˙ O2peak test. This comprises a steady-state cycle to fatigue at a supramaximal power output corresponding to 105% of Wpeak, previously reported to elicit V˙ O2peak values no different from that determined during a ramp incremental test performed 5 min previously (55). Participants were asked to maintain a pedaling cadence of 90 –100 rpm until volitional fatigue, with the test terminated when cadence dropped below 80 rpm. Expired gases were analyzed every 15 s using a custom-made metabolic cart. The gas analyzers (S-3lA/II and CD-3A analyzers; Ametek, Edinboro, PA) were calibrated using known gas concentrations before each test (20.93% O2 and 0.04% CO2; and 16.10% O2 and 4.17% CO2; BOC Gases). V˙ O2peak was defined as the average of the two highest consecutive values reached during the test. Recovery Interventions Exactly 5 min after completing each training session, participants performed their assigned recovery intervention for 15 min. Seated (with legs fully extended), participants were immersed in water up to their umbilicus (COLD: 10°C) or rested on a laboratory bed (CON: 23°C). The COLD condition was performed in an inflatable bath (iBody; iCool Sport, Miami, QLD, Australia), and water temperature was maintained with a cooling/heating unit (Dual Temp Unit; iCool Sport) as previously described (12). We have previously reported the application of this CWI protocol after the same exercise protocol to reduce intramuscular (4 cm) temperature from 37.1 to 33.6°C following an identical exercise session (12). Because of the nature of water immersion per se, a hydrostatic pressure of ~20 –30 mmHg (71) would have been exerted on the COLD participants during recovery. There were no observed or reported signs of shivering during CWI for all participants, at all time points. Muscle Analyses: Biopsy Trial (Pre- vs. Post-Rec vs. 3 h) Preparation of whole muscle lysates for Western blotting. A 10- to 15-mg piece of frozen muscle was added to ice-cold homogenizing buffer (37.5 l/mg tissue; 20 mM Tris, 137 mM NaCl, 1% Triton-X, 10% glycerol, 2.7 mM KCl, 1 mM MgCl, 1 mM EDTA, 1 g/ml aprotinin, 1 g/ml leupeptin, 1 mM benzamedine, 1 mM Na3VO4, 1 mM NaF, 5 mM sodium pyrophosphate, 1 mM dithiothreitol, and 1 mM phenylmethanesulfonyl fluoride; adjusted to pH 7.4) and homogenized on ice using a motorized pestle. The whole muscle lysate was used for Western blotting without centrifugation (46). Protein concentration of the lysate was determined using a commercially available colorimetric assay (Bio-Rad, Hercules, CA), using bovine serum albumin (BSA) as the standard. All samples were diluted with distilled water to a standard concentration (1.5 g/l) and further diluted with 2 Laemmli buffer (125 mmol/l Tris·HCl, pH 6.8, 4% SDS, 20% glycerol, 0.015% bromophenol blue, and 10% -mercaptoethanol). The resultant lysate was split equally into two parts: one unboiled and the other boiled at 95°C for 5 min. Both lysate fractions were subsequently stored at 80°C for future analyses. Western blot analysis. Optimization gels were run for all antibodies to determine optimal conditions for Western blot detection (i.e., loading volume and boiled vs. unboiled lysate). Muscle lysates were loaded equally (15 g) and separated on 10% self-cast SDS-PAGE gels by means of electrophoresis, at 100 V for 1.5–2 h (Bio-Rad, Mississauga, ON, Canada). Unboiled muscle lysate was used for all proteins except p-p53Ser15, in which samples were reboiled at 95°C for 3 min before loading. Once resolved, gels were transferred wet onto polyvinylidene fluoride membranes in transfer buffer (25 mM Tris, 192 mM glycine, 20% methanol, and 0.01% SDS) at 100 V for 90 min. Membranes were then blocked with 5% skim milk in Tris-buffered saline (TBST: 150 mM NaCl, 20 mM Tris, and 0.1% Tween 20, pH 7.4) for 1 h at room temperature. After 5 5-min washes in TBST, the membranes were incubated overnight (4°C with gentle agitation) with monoclonal primary antibodies (Cell Signaling Technology), diluted 1:1,000 in 5% BSA or skim milk, against phosphorylated-AMPKThr172 (product no. 2531; Cell Signaling Technology), p38 mitogen-activated protein kinase (p38 MAPKThr180/Tyr182; no. 9211; Cell Signaling Technology), and p53Ser15 (no. 9284; Cell Signaling Technology). The following morning, membranes were again washed 5 5 min in TBST and incubated in the appropriate species-specific horseradish peroxidase-conjugated secondary antibodies (goat anti-rabbit IgG, NEF812001EA; PerkinElmer, Waltham, MA) at room temperature for 90 min, diluted 1:10,000 (1:5,000 for p-AMPK only) in 5% skim milk and TBST. After a further 5 5-min washes in TBST, membranes were exposed to a chemiluminescent liquid (Clarity Western ECL Substrate; Bio-Rad, Hercules, CA) and visualized using a VersaDoc 4000 MP imaging system (Bio-Rad). Band densities were determined using Image Laboratory 5.1 software (Bio-Rad). Membranes were then stained with 0.1% Coomassie R-350 (PhastGel Blue R; GE Healthcare) as previously described (69), thereby providing a loading and transfer control. Comparative samples for each participant were loaded into the same gel, and one lane for each gel was reserved for an internal standard to account for intergel variability. Raw blot density was normalized to the internal standard and Coomassie stain before analysis.
Total RNA isolation. Approximately 20 –30 mg of frozen muscle were added to 1 g of zirconia/silica beads (1.0 mm; Daintree Scientific, St. Helens, TAS, Australia) and 800 l of commercially available TRIzol reagent (Invitrogen, Carlsbad, CA) and homogenized using an electronic homogenizer (FastPrep FP120 Homogenizer; Thermo Savant). Samples were centrifuged at 13,000 rpm for 15 min (4°C) to pellet cell debris. The upper homogenate was then removed and pipetted into 250 l chloroform (Sigma-Aldrich, St. Louis, MO) and again centrifuged at 13,000 rpm for 15 min (4°C). Without disturbing the interphase, the top phase was pipetted into a fresh Eppendorf containing 400 l of 2-isopropanol alcohol (SigmaAldrich) and 10 l of 5 M NaCl and stored at 20°C overnight to allow for RNA precipitation. The following morning the sample was centrifuged at 13,000 rpm for 20 min (4°C), following which the majority of the isopropanol was aspirated. The remaining RNA pellet was washed once with 75% ethanol made with diethylpyrocarbonatetreated (DEPC) H2O (Invitrogen Life Sciences) and centrifuged at 9,000 rpm for 8 min (4°C). Ethanol was aspirated off, and the pellet was resuspended in 5 l of heated DEPC-treated H2O. RNA concentration was quantified spectrophotometrically (NanoDrop 2000; Thermo Fisher Scientific, Wilmington, DE) at 230 (A230), 260 (A260), and 280 (A280) nm, with an average yield of 1,251.9 466.7 ng/l, an A260/280 ratio of 1.78 0.11, and an A260/230 ratio of 1.14 0.38. RNA was stored at 80°C until further analysis. Real-time reverse transcriptase-PCR. One microgram of RNA was reverse transcribed into cDNA using a thermal cycler (S1000 Thermal Cycler; Bio-Rad) and a commercially available kit (iScript cDNA Synthesis Kit; Bio-Rad, Melbourne, VIC, Australia) with random hexamers and oligo(dT)s, according to the manufacturer’s instructions. All samples and reverse transcriptase (RT) negative controls were run together to prevent technical variation. “Real-time” PCR reactions (total volume 10 l) were performed with iTaq Universal SYBR Green Supermix (Bio-Rad, Hercules, CA) as the fluorescent agent. The following cycling patterns were used: initial denaturation at 95°C for 3 min, 40 cycles of 95°C for 15 s and 60°C for 60 s (Mastercycler RealPlex2 ; Eppendorf, Hamburg, Germany). Forward and reverse primers for target genes were designed using sequences obtained from GenBank (Table 2). All samples were run in duplicate with negative controls, using an automated pipetting system (epMotion 5070; Eppendorf). To account for the efficiency of RT and initial RNA concentration, the mRNA abundance of the housekeeping genes glyceraldehyde-3-phosphate dehydrogenase (GAPDH), TATA-binding protein (TBP) and 2-microglobulin (B2M) was quantified, and target genes were normalized to the geometric mean of all three. Relative changes in mRNA abundance were calculated as described previously (31).
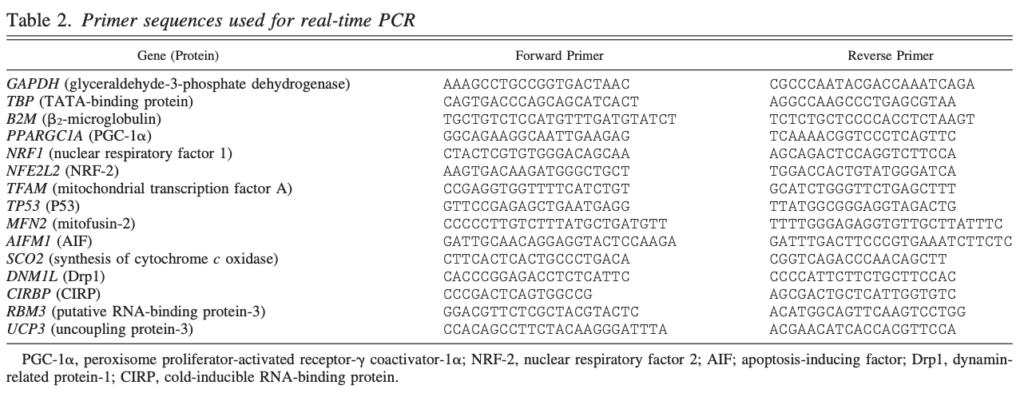
Muscle Analyses: SIT Intervention (Pre vs. Post) Preparation of permeabilized skeletal muscle fibers. A small section (~15–20 mg) of fresh muscle was analyzed for mitochondrial respiration pretraining and posttraining. The sample was immediately placed into a cold biopsy preservation solution [BIOPS; 2.77 mM CaK2EGTA, 7.23 mM K2EGTA, 5.77 mM Na2ATP, 6.56 mM MgCl2, 20 mM taurine, 50 mM K-4-morpholinoethanesulfonic acid (MES), 15 mM Na2phosphocreatine, 20 mM imidazole, and 0.5 mM dithiothreitol (DTT), pH 7.1 (52)] and subsequently mechanically separated using forceps. Fibers were permeabilized by gentle agitation (30 min at 4°C) in a solution of BIOPS containing 50 g/ml of saponin and washed (3 7 min at 4°C) by gentle agitation in the respiration medium solution [MiR05; 0.5 mM EGTA, 3 mM MgCl2, 60 mM K-lactobionate, 20 mM taurine, 10 mM KH2PO4, 20 mM HEPES, 110 mM sucrose, and 1% BSA, pH 7.1 at 37°C (52)]. This method selectively permeabilizes the cellular membrane leaving the mitochondria intact, allowing for “in situ” measurements of mitochondrial respiration. High-resolution respirometry. After washing was completed, fibers were weighed (~3– 4 mg wet wt) and assayed in duplicate in a high-resolution respirometer (Oxygraph-2k; Oroboros Instruments, Innsbruck, Austria), with each chamber containing 2 ml of MiR05. Oxygen concentration (nmol/ml) and oxygen flux (pmol·s 1 ·mg 1 ) were measured at 37°C and recorded using DatLab software (version 5; Oroboros Instruments), corrected to instrumental background oxygen flux. Each chamber was continually reoxygenated by direct syringe injection of O2 into the chamber to allow for maintenance of O2 concentration (275– 450 nmol/ml) to avoid a potential oxygen diffusion limitation. Mitochondrial respiration measurements were taking using a substrate-uncoupler-inhibitor titration (SUIT) protocol. First, the substrates pyruvate (final chamber concentration; 2 mM) and malate (5 mM) were added in the absence of adenylates for measurement of leak respiration (L) with electron entry through complex I (CI; CIL). Next, adenosine diphosphate (ADP) was added (5 mM) for measurement of maximal oxidative phosphorylation (OXPHOS) capacity (P) with electron input through CI (CIP), followed by addition of succinate (10 mM) for measurement of P with electron supply through CI and complex II (CII) combined (CI&IIP). This state provides convergent electron input to the Q-junction through CI (NADH provided by malate and pyruvate) and CII (FADH2 provided by succinate) and supports maximal mitochondrial respiration by reconstruction of the citric acid cycle function. Cytochrome c oxidase (10 M) was next added to assess outer mitochondrial membrane integrity; increases in O2 flux 10% after cytochrome c oxidase addition indicated compromised membrane integrity, in which data were excluded. A series of stepwise carbonyl cyanide 4-(trifluoromethoxy)phenylhydrazone titrations (FCCP, 0.75–1.5 M) were performed, to determine maximal uncoupled respiration and electron transport system capacity (E), with convergent electron input through CI and CII (CI&IIE). Rotenone (0.5 M), an inhibitor of CI, was added next to determine E with electron input through CII alone (CIIE). Antimycin A (2.5 M), an inhibitor of complex III (CIII), was then added to measure residual oxygen consumption capacity (ROX); this was used to correct all respiration values. Mitochondrial respiration measures represent an average of the technical duplicates (i.e., average of both chambers), expressed in both absolute oxygen flux (mass-specific mitochondrial respiration) and corrected to citrate synthase activity (mitochondriaspecific respiration). Complete mitochondrial respiration data sets were available for 12 participants (CON, n 6; COLD, n 6) as a result of malfunctions with the respirometer and/or poor muscle sample quality. Preparation of whole muscle lysates and Western blot analyses. Preparation of the whole muscle lysate was performed in the same manner as that for the biopsy trial. The whole muscle lysate was used for both enzyme activity assays and Western blotting, without centrifugation. Western blots were performed with unboiled muscle lysate for all proteins except p53, in which samples were reboiled at 95°C for 3 min before loading. Membranes were incubated overnight with monoclonal primary antibodies (1:500 –5,000 in 5% skim milk) against p53 (no. 2527; Cell Signaling Technology) and PGC-1 (ST1202; Merck Millipore, Billerica, MA). Secondary antibodies [goat anti-mouse IgG (NEF822001EA; PerkinElmer) for PGC-1] diluted 1:7,000 –30,000 in 5% skim milk and TBST. All other Western blot procedures were identical to those detailed in the biopsy trial methodology. Citrate synthase activity. The maximal enzyme activity of citrate synthase (CS) was determined using the lysate prepared for Western blot analyses and analyzed on a spectrophotometer (Bio-Rad). Each well contained a 50-fold dilution of lysate (2 mg/ml), 3 mM acetylCoA, 1 mM dystrobrevin- (DTNB), and 10 mM oxaloacetate (OAA), made to volume (250 l) with Tris buffer (100 mM). Reactions were analyzed over a 3-min period at 30°C, with absorbance (412 nM) recorded every 15 s. Samples were analyzed in triplicate, with enzyme activity expressed as moles per hour per kilogram of protein (M·h 1 ·kg protein 1 ). Statistical Analyses Data are reported in the text as means SD, unless otherwise stated. Comparisons between conditions were analyzed using a twoway general linear model (ANOVA) with repeated measures for time, where the within-subject factor was time (biopsy trial, Pre vs. PostRec vs. 3-h; training intervention, Pre vs. Post) and the betweensubject factor was condition (CON vs. COLD). Where significant time or interaction (time condition) effects were found, multiple pairwise comparisons were evaluated by Welch’s t-test, corrected to the false discovery rate method (19). The level of significance for all data was set at P 0.05. The above analyses were performed using SPSS Statistics version 20 (IBM). To compliment the statistical hypothesis testing, effect sizes (ES) were calculated to assess the magnitude of observed effects. Cohen’s conventions for effect size (Cohen’s d 90% confidence intervals) were used for interpretation, where ES 0.2, 0.5, and 0.8 are considered as small, medium and large, respectively. Raw Western blot densitometry data (corrected to internal standard and Coomassie stain) and changes in mRNA abundance (RT-PCR data) were used for statistical analyses. For graphical purposes, baseline Western blot and RT-PCR values were normalized to 1.0, such that Post-Rec and 3-h values correspond to fold change from Pre values. RESULTS SIT Session and Training Both groups performed similar volumes of total work during the single session of SIT, 67.5 11.8 (CON) and 65.3 10.1 kJ (COLD) (P 0.05). Similarly, both groups performed similar volumes of training over the 6 wk, 1,518.7 180.9 and 1,487.0 242.4 kJ for CON and COLD, respectively (P 0.05). There was 98% adherence to the training program, with two participants in the CON group and three participants in the COLD group missing one training session each because of illness. No differences were observed for the characteristics listed in Table 1 (P 0.05), demonstrating effective randomization of participants into the two conditions. Muscle Analyses: Biopsy Trial Western blots. Significant effects of time were observed for the phosphorylation (p-) of p38 MAPKThr180/182 (P 0.029) and p-AMPKThr172 (P 0.002). Specifically, p-p38 MAPKThr180/182 was elevated postrecovery (P 0.029, ES 1.00 0.70) but returned to basal levels by 3 h postrecovery (P 0.487). There was a similar increase in p-AMPKThr172 postrecovery (P 0.002, ES 0.78 0.51), which remained significantly elevated 3 h postrecovery (P 0.006, ES 0.77 0.40). There were no interaction effects for either p-p38 MAPKThr180/182 (P 0.901) or p-AMPKThr172 (P 0.472; Fig. 1). There was a significant effect of time for p-p53Ser15 (P 0.007). Specifically, p-p53Ser15 was elevated postrecovery (P 0.011, ES 1.54 1.15) and remained significantly elevated 3 h postrecovery (P 0.011, ES 2.39 1.70). There was no interaction effect for p-p53Ser15 (P 0.142). However, there were large effects for the change in p-p53Ser15 content between conditions (COLD vs. CON) at both postrecovery (2.7-fold vs. 1.5-fold; ES 1.68 1.79) and 3 h postrecovery (3.7-fold vs. 1.7-fold; ES 2.69 2.78; Fig. 1). mRNA. There were no effects of time for the housekeeping genes GAPDH (P 0.668), TBP (P 0.748), and 2M (P 0.193). A significant effect of time (P 0.001) was observed for PGC-1 mRNA, with post hoc analysis revealing that it was significantly elevated 3 h postrecovery (P 0.001, ES 8.49 1.77). However, no interaction effect was observed for PGC-1 mRNA (P 0.738). There were no time or interaction effects for the mRNA content of nuclear respiratory factor 1 (NRF-1; P 0.212 and 0.812, respectively), nuclear respiratory factor 2 (NRF-2; P 0.492 and 0.645, respectively), or mitochondrial transcription factor (TFAM; P 0.318 and 0.467, respectively; Fig. 2A). There were no main effects of time for the mRNA content of P53 (P 0.062), or the p53 downstream targets mitofusin-2 (MFN2; P 0.335), dynamin-related protein-1 (DRP1; P 0.994), synthesis of cytochrome c oxidase (SCO2; P 0.274), or apoptosis-inducing factor (AIF; P 0.517). An interaction effect was demonstrated for MFN2 mRNA (P 0.033), but when corrected to the false discovery rate, the critical significance level was not achieved. There were no interaction effects for P53 (P 0.707), DRP1 (P 0.279), SCO2 (P 0.214), or AIF (P 0.078) mRNA. At the 3-h-postrecovery time point, there were moderate effects for the change in mRNA content of MFN2 (1.2-fold vs. 0.8-fold; ES 0.53 0.40), AIF (1.3-fold vs. 0.9-fold; ES 0.63 0.65), and SCO2 (1.3- fold vs. no change; ES 0.70 0.80) between conditions (Fig. 2B). No time or interaction effects were observed for the mRNA content of cold-inducible RNA-binding protein (CIRP; P 0.475 and 0.168, respectively), RNA-binding motif protein-3 (RBM3; P 0.151 and 0.262, respectively), or uncoupling protein-3 (UCP3; P 0.245 and 0.490, respectively). However, there was a moderate effect (ES 0.50 0.57) for the change in RBM3 mRNA content between conditions 3 h postrecovery (1.3-fold vs. no change; Fig. 2C). Muscle Analyses: Training Intervention Western blots. There were no time or interaction effects for PGC-1 (P 0.437 and 0.751, respectively) or p53 (P 0.304 and 0.446, respectively) content (Fig. 3). CS activity and mitochondrial respiration. There were no time (10.0 23.8% increase; P 0.232) or interaction (P 0.656) effects for changes in maximal citrate synthase activity as a result of training (Fig. 4). There were no interaction effects for all mass-specific respiration measures (P 0.05). However, there was a significant time effect for CI&IIE (P 0.013, ES 0.82 0.48), which increased 21.6 24.8% after 6 wk of SIT. Similar mean percentage changes were observed for the change in CIP (23.8 47.5%; P 0.181, ES 0.37 0.49), CI&IIP (13.4 19.8%; P 0.172, ES 0.43 0.35), and CIIE (30.6 47.5%; P 0.546, ES 0.61 0.53) as a result of training, but these were not significant because of the considerable individual variability observed (Fig. 5A). When normalized to CS activity (mitochondrial-specific respiration), there were no interaction effects for all measures (P 0.05). There were no time effects for CIL (P 0.648), CIP (P 0.453), or CI&CIIP (P 0.656), and small effects (with no effect of time) for the change in CI&IIE (11.4 24.1%; P 0.121, ES 0.33 0.47) and CIIE (21.8 49.7%; P 0.775, ES 0.31 0.58) as a result of training (Fig. 5B).
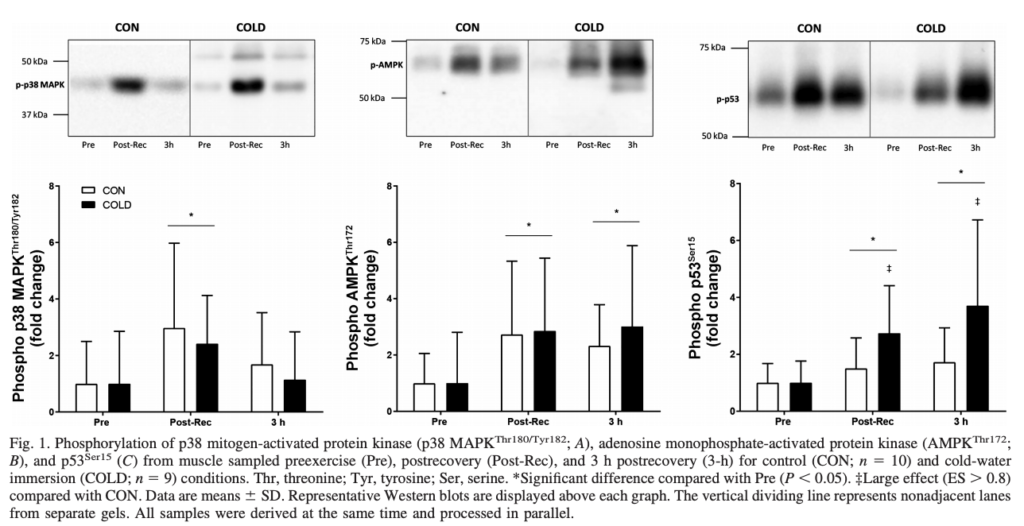
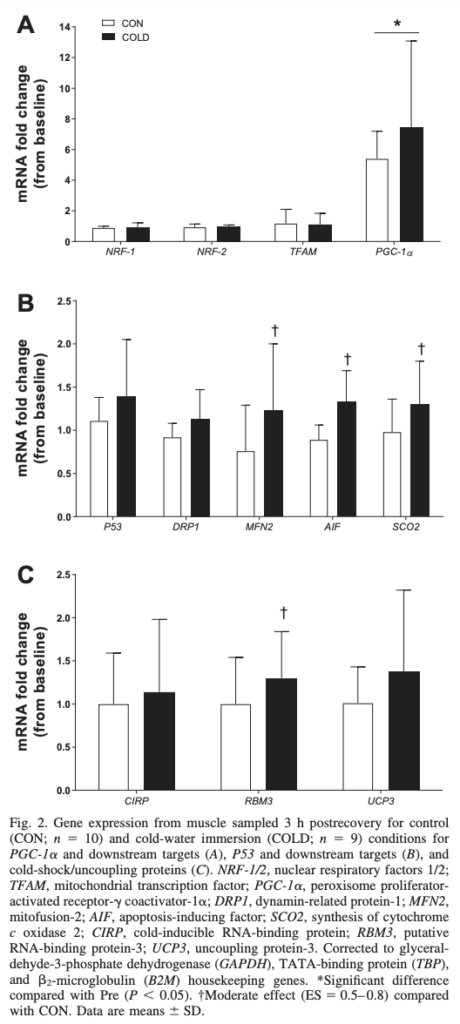
Performance Measures GXT and V˙ O2peak. Peak power output during the GXT increased by 5.8 5.7 and 6.1 5.6% in the CON and COLD groups, respectively (time effect; P 0.001, ES 0.31 0.12). Similarly, V˙ O2peak increased significantly as a result of training (main effect of time; P 0.002, ES 0.61 0.28), with a 9.6 6.7% increase in the CON group and a 7.6 11.0% increase in the COLD group. There were no main interaction effects for peak power output (P 0.979) or V˙ O2peak (P 0.633; Fig. 6). As a result of training, the lactate threshold increased from 162.1 26.7 to 171.3 36.6 W for the CON group and from 155.8 54.8 to 160.2 59.8 W for the COLD group, with no main effect of time (P 0.074) or interaction (P 0.494). Time trials. The time required to finish the TT2 decreased after training by 3.3 4.5 and 2.4 3.1% in the CON and COLD groups, respectively (time effect; P 0.010, ES 0.36 0.21). TT2 mean power correspondingly increased from 261.3 45.0 to 283.5 46.1 W for the CON group and from 248.1 66.4 to 263.7 59.2 W for the COLD group (time effect; P 0.023, ES 0.34 0.21). There were no effects of time for TT20 duration (P 0.224) or mean power (P 0.208). Furthermore, there were no interaction effects for TT2 duration (P 0.699) and mean power (P 0.666), or TT20 duration (P 0.889) and mean power (P 0.703) (Fig. 7). DISCUSSION The main finding of this study was that contrary to expectations, CWI administered after sprint interval exercise had limited effects on exercise-induced mitochondrial biogenesis, changes in mitochondrial content or function, and measures of endurance exercise performance. A novel finding of this study was that p53 phosphorylation is increased following a single session of SIT; however, this response did not translate into training-induced increases in the content of this protein after 6 wk of SIT. In contrast to previous reports of greater increases in PGC-1 mRNA following a single exposure to postexercise CWI (34) and greater increases in PGC-1 protein content following regular postexercise CWI (33), we observed no differences between conditions in the present study. Six weeks of SIT significantly increased peak aerobic power, V˙ O2peak, maximal uncoupled respiration (through complexes I and II), and 2-km time trial performance. However, regular CWI performed after each training session had no effect on changes in these parameters, consistent with the lack of an effect of CWI on markers of mitochondrial biogenesis following a single exercise session. Although these observations suggest that CWI is not detrimental to endurance adaptations in response to 6 wk of SIT, they question whether CWI following SIT is an effective strategy to promote mitochondrial biogenesis and improvements in endurance performance. Consistent with previous work following high-intensity interval and continuous running (7), this study provides the observation that p53Ser15 phosphorylation is increased in human skeletal muscle following a single session of SIT (4 30-s all-out cycling efforts; Fig. 1). Additionally, we observed that this response is unaltered by CWI. As shown in a number of different cell and tissue types, p53 plays an important role in regulating mitochondrial content and oxidative capacity (5). In
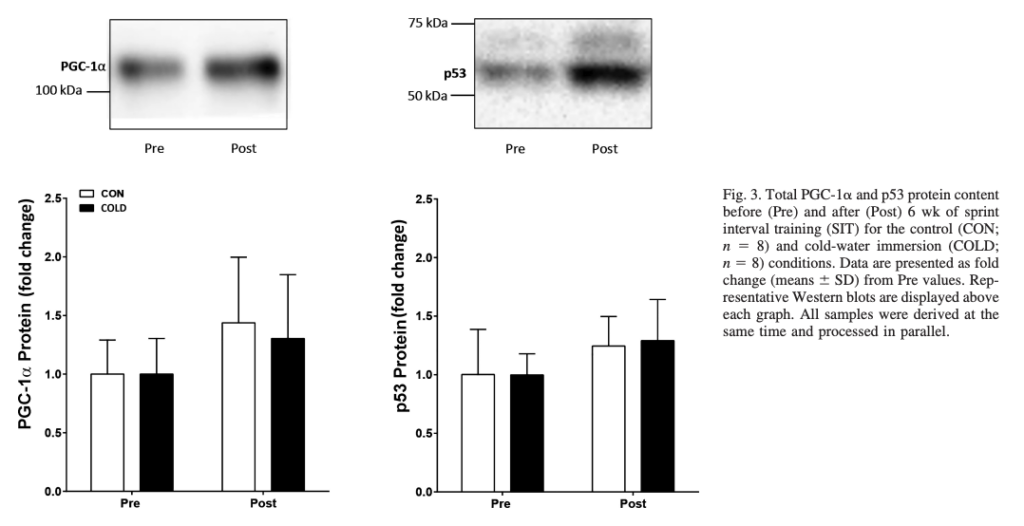
contrast to the response to other nongenotoxic stresses, such as fluctuations in oxygen or carbohydrate availability (8, 9), the cold stress imposed by postexercise CWI had no significant effect on p53 phosphorylation. Given the paucity of research investigating the acute effects of exercise on the p53 pathway, it is possible that the 3-h-postrecovery time point may not have captured the maximal increase in the phosphorylation of this protein. Nonetheless, the large difference in p53 phosphorylation between conditions 3 h postrecovery (increase of 3.7-fold for COLD vs. 1.7-fold for CON) suggests that further research is warranted to investigate the effects of CWI on the p53 pathway. To further test the hypothesis that CWI may augment exercise-induced p53 phosphorylation and, consequently, stability (18), a number of p53 target genes were also investigated
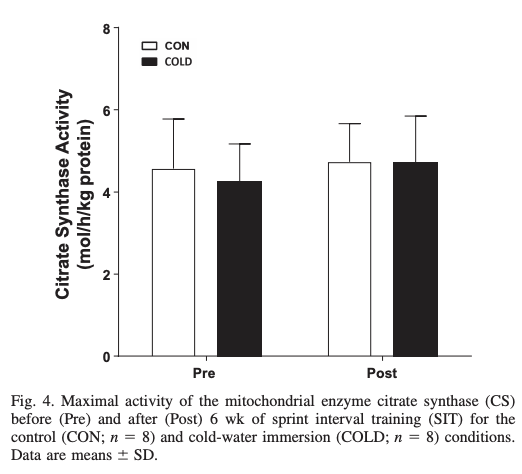
(Fig. 2B). Two proteins central to mitochondrial remodeling are the fusion protein Mfn2 and the fission protein Drp1, both of which are activated by p53 (39, 68). Mfn2 is a mitochondrial GTPase that plays an important role in mitochondrial fusion, therefore contributing to the maintenance of the mitochondrial network (4). In contrast to the increase in MFN2 mRNA following a single bout of submaximal intensity continuous cycling (1 h at 60% Wmax; 36, 62), there was no change in both conditions in the present study. Similarly, there was no change in DRP1 mRNA in both conditions in the present study, consistent with previous research demonstrating unaltered protein content following 2– 4 wk of high-intensity interval training (HIT; 27, 28, 51). Other downstream targets of p53, AIF and SCO2 mRNA, were unaltered in the CON condition in the present study, consistent with the few published studies available (42, 57). We add that this response was not influenced by postexercise CWI. Postexercise CWI also had no additional effect on the exercise-induced increases in AMPK and p38 MAPK phosphorylation, which has previously been reported following a single session of high-intensity cycling (24, 40). The prolonged increase in AMPK phosphorylation (up to 3 h postrecovery) observed in the present study was most likely due to glucose deprivation following an extended fast (~12 h by the 3-hpostrecovery biopsy) and the completion of an intense exercise session (15). Contrary to expectations, CWI did not augment p38 MAPK phosphorylation, despite hypothermic stress being a well-accepted upstream activator (26). This can possibly be attributed, in part, to the already large exercise-induced increase in the level of p38 MAPK phosphorylation observed [~250 –300% compared with previous reports of an ~140% increase (24)]; this may have meant that there was no augmented response to the additional stress of CWI. Alternatively, the 3-h-postrecovery biopsy may not have adequately captured the time course of changes in the AMPK and p38 MAPK
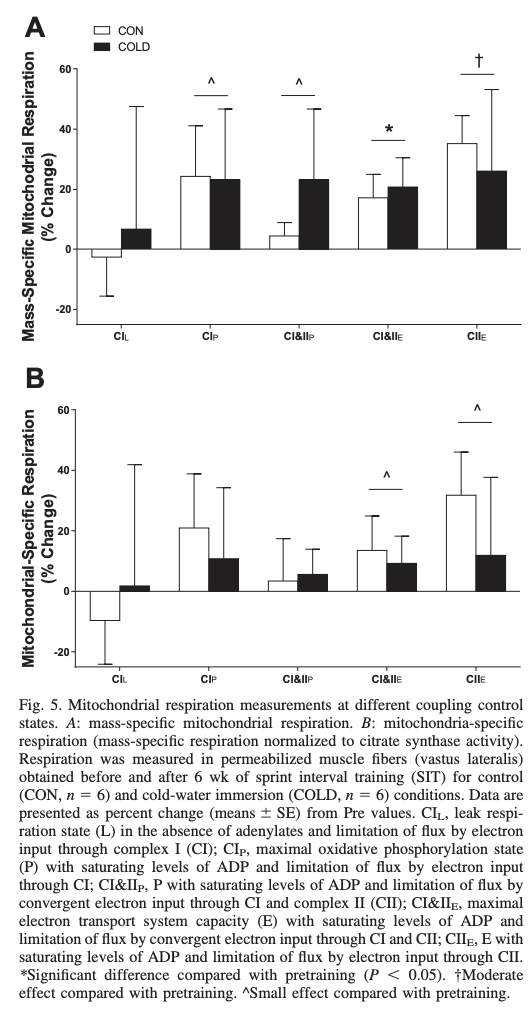
signaling pathways following postexercise CWI. However, consistent with links between activation of p38 MAPK and AMPK and downstream p53Ser15 phosphorylation (7), CWI had no additional effect on the phosphorylation of these kinases following a single session of SIT. Both energy-dependent kinase signaling (AMPK) and stressdependent (p38 MAPK) kinase signaling (2, 35) have been implicated in PGC-1 activation following HIT (41), a response that was supported in the present study. Similar to previous research (40), we observed an increase in PGC-1 mRNA following a single session of SIT (Fig. 2A). However, postexercise CWI had no additional influence on the increase in PGC-1 mRNA, consistent with the lack of an influence on its upstream activators p38 MAPK and AMPK. This contrasts with previous reports that postexercise CWI (8 –10°C for 10 –15 min; 34, 37) and cold ambient exposure (4°C for 3– 4 h; 61, 62) augment PGC-1 mRNA levels compared with a passive room temperature control. However, certain methodological differences may explain the inconsistencies between these studies and the present study. For example, it is unlikely that 15 min of CWI would have elicited a shivering response and/or a reduction in core temperature of the same magnitude as the 3– 4-h cold exposure used in the two studies from Slivka (61, 62). In regard to the studies by Ihsan et al. (34) and Joo et al. (37), these inconsistencies could be explained by the mode and intensity of exercise (and thus the metabolic stimulus) performed before CWI, the temperature and duration of CWI, differences in nutritional status of participants between studies, and/or differences in RT-PCR methodology. This may be further compounded by the fact that our participants were fasted, which may similarly enhance AMPK and p38 MAPK phosphorylation and subsequent PGC-1 activation (6). To provide further insight into the effects of regular postexercise CWI on potential cellular mechanisms modulating adaptation to SIT, the protein content of PGC-1 following the training period was also measured. No difference was observed between the CON and COLD conditions, consistent with the similar changes in PGC-1 mRNA observed after a single session of SIT with and without CWI. This is consistent with the nonsignificant ~1.2-fold increase in PGC-1 protein content observed when CWI was used regularly after a highintensity-running training period (33). Although PGC-1 protein has been reported to increase (~1.5 fold) following cold-air exposure (4°C for as little as 4 days) in rodent skeletal muscle (13, 49), these increases were likely the result of shivering thermogenesis (49) and extended cold exposure (i.e., 4°C, 24 h/day for 4 –5 wk; 13), which were not characteristic of the CWI protocol used in the present study. The present data therefore suggest that CWI has no effect on SIT-induced PGC-1 protein accumulation in humans, and continued work in this area (including potential CWI-induced alterations in PGC-1 posttranslational modification and nuclear content) is needed to help clarify the above-mentioned inconsistencies. A novel aspect of this study was to directly assess for the first time the effect of regular postexercise CWI on traininginduced improvements in mitochondrial respiration in permeabilized muscle fibers. Despite a smaller than expected increase for the CON group in the present study, there was a ~13% increase in maximal mass-specific respiration (CI&IIP) when participants were pooled, comparable to previous reports in participants of a similar training status (28). The smaller improvements reported in the present study, compared with Granata et al. (28), may be explained by the number of all-out bouts performed, differences in age and training status, and the large variability typically associated with respiration measures. However, consistent with the similar response for markers of mitochondrial adaptation (i.e., PGC-1 and p53 protein content), regular CWI had no influence on training-induced changes in mass-specific mitochondrial respiration. To determine whether the increases in mass-specific respiration were qualitative or quantitative, mass-specific respiration was corrected to CS activity (to calculate mitochondriaspecific respiration). Located exclusively in the mitochondria (65), CS is an enzyme commonly used in exercise-training studies as a biomarker of mitochondrial content (11, 38).
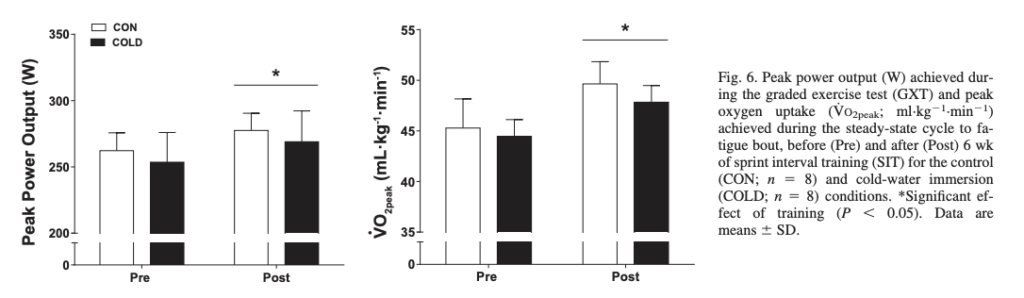
Similar to the observations for mass-specific respiration and consistent with similar training-induced increases in PGC-1 and p53 protein content, CWI had no effect on changes in mitochondria-specific respiration. As such, and contrary to our hypothesis, these data suggest that CWI does not serve to augment increases in mitochondrial respiration in response to SIT. Further research is warranted to investigate whether CWI can augment training-induced mitochondrial adaptations, especially when combined with a larger training volume that will induce more robust increases in CS activity/mitochondrial content (11). Another novel aspect of this study was to investigate changes in the mRNA content of the cold-shock proteins CIRP and RBM3, and UCP3, following postexercise CWI (Fig. 2C). It is well accepted that transcription and translation are suppressed in response to cold stress, accompanied by a reduction in RNA degradation and alternate splicing of pre-mRNA (3, 63, 73). Despite their integral roles in mRNA stabilization and global protein synthesis under cold stress (20, 63), CIRP and RBM3 mRNA were unchanged following postexercise CWI. Previously reported to reduce intramuscular temperature by ~3°C (12), the CWI protocol used in the present study induced a small (~30%) yet nonsignificant increase in RBM3 mRNA 3 h after CWI. However, it is possible that a more drastic reduction in muscle temperature (~5°C) is needed for a significant increase in RBM3 mRNA (20). Similarly, CIRP mRNA was unchanged 3 h following postexercise CWI. The expression of CIRP mRNA, however, has been reported to peak between 6 and 24 h after mild (32°C) cold exposure in mouse fibroblasts (48), which may explain the lack of response 3 h after postexercise CWI. UCP3, an uncoupling protein expressed predominantly in skeletal muscle (60), has recently been implicated as an integral mediator of mammalian thermogenesis during cold exposure (54). In the present study, UCP3 mRNA was unchanged 3 h following postexercise CWI (Fig. 2C), consistent with the response reported by Slivka et al. (62). It is possible that the CWI protocol used in the present study did not provide an adequate cold stress to elicit an increase in UCP3 mRNA. Another potential explanation for the subtle effect of postexercise CWI on CIRP, RBM3, and UCP3 mRNA is the exercise stimulus itself, as a more pronounced increase in gene expression may have occurred without a preceding exercise stimulus. An important applied aspect of this research was to determine whether regular CWI had any effect on exercise performance. Although it has previously been suggested that CWI may be detrimental to training-induced changes in exercise performance (74), this study provides evidence that regular CWI following sprint interval cycling has no detrimental (or advantageous) influence on physiological and performance markers of endurance adaptations, including time trial performance, the lactate threshold, V˙ O2peak, and peak aerobic power. These findings support the absence of a significant difference between conditions for the measured markers of mitochondrial biogenesis (i.e., p53, PGC-1, CS activity, etc.). It is important to note that V˙ O2peak, peak aerobic power, and time trial performance all increased following SIT, without concurrent increases in molecular markers (i.e., PGC-1 protein content, mitochondrial respiration, or CS activity). Although these molecular markers have been associated with improved V˙ O2peak (41), many other factors could have contributed to the increase in performance markers as a result of SIT, including alterations in hemodynamic (16, 17) and cardiorespiratory (14, 45, 66) factors, as well as improvements in substrate transport
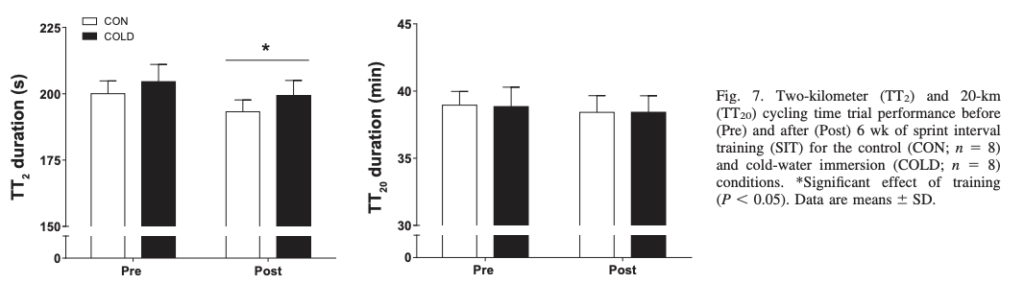
and utilization (14, 17, 25). Nonetheless, these performance data support the two published studies investigating the effects of regular CWI following cycling training, whereby CWI performed regularly during 3– 4 wk of cycling training did not impair performance adaptations in competitive cyclists (29) or recreationally active males (1). Given that the participants in the present study were considerably less trained in comparison, this suggests that training status does not influence these effects. Finally, these data, along with those of Halson et al. (29), do not support concerns of CWI being detrimental to adaptations to endurance training (32, 74). Perspectives and Significance The most important finding from the present study was that regular CWI following sprint interval cycling has no advantageous (or detrimental) influence on markers of endurancerelated training adaptations and cycling performance. This study provides evidence that p53 phosphorylation is increased following a single session of SIT; however, this did not translate into a greater increase in its content following 6 wk of SIT, suggesting that this signaling response does not accurately predict training responses. Contrary to our hypothesis, a number of molecular markers associated with skeletal muscle metabolic adaptations and mitochondrial biogenesis were not altered by postexercise CWI compared with a passive control. For example, CWI had no additional influence on the exerciseinduced increases in p38 MAPK or AMPK activation, consistent with the lack of response of their downstream targets PGC-1 and p53. Given the inconsistencies in molecular responses between the present study and those reported with CWI following high-intensity interval exercise (12, 15), future research incorporating larger sample sizes and/or additional biopsy time points may help clarify the effects of CWI on the signaling pathways and training adaptations associated with SIT. These findings not only refute previous suggestions that exercise-induced training benefits may be counteracted by possible CWI-induced long-term detrimental effects on muscular adaptation but also question whether postexercise CWI following sprint interval cycling is an effective strategy to promote mitochondrial biogenesis and improvements in endurance performance. ACKNOWLEDGMENTS We acknowledge Dr. Jujiao Kuang, Dr. Jon Bartlett, and Karen Hill for help with data collection and analysis and critical review of the manuscript. Dr. Mitch Anderson and Dr. Andrew Hodder are also acknowledged for the collection of muscle samples. GRANTS This work was partly funded by an Exercise & Sports Science Australia Research Grant. DISCLOSURES No conflicts of interest, financial or otherwise, are declared by the authors. AUTHOR CONTRIBUTIONS J.R.B. and D.J.B. conceived and designed research; J.R.B. performed experiments; J.R.B. and D.J.B. analyzed data; J.R.B., A.P., and D.J.B. interpreted results of experiments; J.R.B. prepared figures; J.R.B. drafted manuscript; J.R.B., A.P., and D.J.B. edited and revised manuscript; J.R.B., A.P., and D.J.B. approved final version of manuscript.
REFERENCES 1. Aguiar PF, Magalhaes SM, Fonseca IA, da Costa Santos VB, de Matos MA, Peixoto MF, Nakamura FY, Crandall C, Araujo HN, Silveira LR, Rocha-Vieira E, de Castro Magalhaes F, Amorim FT. Postexercise cold water immersion does not alter high intensity interval training-induced exercise performance and Hsp72 responses, but enhances mitochondrial markers. Cell Stress Chaperones 21: 793–804, 2016. doi: 10.1007/s12192-016-0704-6. 2. Akimoto T, Pohnert SC, Li P, Zhang M, Gumbs C, Rosenberg PB, Williams RS, Yan Z. Exercise stimulates Pgc-1alpha transcription in skeletal muscle through activation of the p38 MAPK pathway. J Biol Chem 280: 19587–19593, 2005. doi:10.1074/jbc.M408862200. 3. Ars E, Serra E, de la Luna S, Estivill X, Lázaro C. Cold shock induces the insertion of a cryptic exon in the neurofibromatosis type 1 (NF1) mRNA. Nucleic Acids Res 28: 1307–1312, 2000. doi:10.1093/nar/28.6. 1307. 4. Bach D, Pich S, Soriano FX, Vega N, Baumgartner B, Oriola J, Daugaard JR, Lloberas J, Camps M, Zierath JR, Rabasa-Lhoret R, Wallberg-Henriksson H, Laville M, Palacín M, Vidal H, Rivera F, Brand M, Zorzano A. Mitofusin-2 determines mitochondrial network architecture and mitochondrial metabolism. A novel regulatory mechanism altered in obesity. J Biol Chem 278: 17190 –17197, 2003. doi:10. 1074/jbc.M212754200. 5. Bartlett JD, Close GL, Drust B, Morton JP. The emerging role of p53 in exercise metabolism. Sports Med 44: 303–309, 2014. doi:10.1007/ s40279-013-0127-9. 6. Bartlett JD, Hawley JA, Morton JP. Carbohydrate availability and exercise training adaptation: too much of a good thing? Eur J Sport Sci 15: 3–12, 2015. doi:10.1080/17461391.2014.920926. 7. Bartlett JD, Hwa Joo C, Jeong TS, Louhelainen J, Cochran AJ, Gibala MJ, Gregson W, Close GL, Drust B, Morton JP. Matched work high-intensity interval and continuous running induce similar increases in PGC-1 mRNA, AMPK, p38, and p53 phosphorylation in human skeletal muscle. J Appl Physiol (1985) 112: 1135–1143, 2012. doi:10.1152/ japplphysiol.01040.2011. 8. Bartlett JD, Louhelainen J, Iqbal Z, Cochran AJ, Gibala MJ, Gregson W, Close GL, Drust B, Morton JP. Reduced carbohydrate availability enhances exercise-induced p53 signaling in human skeletal muscle: implications for mitochondrial biogenesis. Am J Physiol Regul Integr Comp Physiol 304: R450 –R458, 2013. doi:10.1152/ajpregu.00498.2012. 9. Berkers CR, Maddocks OD, Cheung EC, Mor I, Vousden KH. Metabolic regulation by p53 family members. Cell Metab 18: 617–633, 2013. doi:10.1016/j.cmet.2013.06.019. 10. Bishop D, Jenkins DG, McEniery M, Carey MF. Relationship between plasma lactate parameters and muscle characteristics in female cyclists. Med Sci Sports Exerc 32: 1088 –1093, 2000. doi:10.1097/00005768- 200006000-00008. 11. Bishop DJ, Granata C, Eynon N. Can we optimise the exercise training prescription to maximise improvements in mitochondria function and content? Biochim Biophys Acta 1840: 1266 –1275, 2014. doi:10.1016/j. bbagen.2013.10.012. 12. Broatch JR, Petersen A, Bishop DJ. Postexercise cold water immersion benefits are not greater than the placebo effect. Med Sci Sports Exerc 46: 2139 –2147, 2014. doi:10.1249/MSS.0000000000000348. 13. Bruton JD, Aydin J, Yamada T, Shabalina IG, Ivarsson N, Zhang SJ, Wada M, Tavi P, Nedergaard J, Katz A, Westerblad H. Increased fatigue resistance linked to Ca2-stimulated mitochondrial biogenesis in muscle fibres of cold-acclimated mice. J Physiol 588: 4275–4288, 2010. doi:10.1113/jphysiol.2010.198598. 14. Burgomaster KA, Howarth KR, Phillips SM, Rakobowchuk M, Macdonald MJ, McGee SL, Gibala MJ. Similar metabolic adaptations during exercise after low volume sprint interval and traditional endurance training in humans. J Physiol 586: 151–160, 2008. doi:10.1113/jphysiol. 2007.142109. 15. Cantó C, Jiang LQ, Deshmukh AS, Mataki C, Coste A, Lagouge M, Zierath JR, Auwerx J. Interdependence of AMPK and SIRT1 for metabolic adaptation to fasting and exercise in skeletal muscle. Cell Metab 11: 213–219, 2010. doi:10.1016/j.cmet.2010.02.006. 16. Cocks M, Shaw CS, Shepherd SO, Fisher JP, Ranasinghe A, Barker TA, Wagenmakers AJ. Sprint interval and moderate-intensity continuous training have equal benefits on aerobic capacity, insulin sensitivity, muscle capillarisation and endothelial eNOS/NAD(P)Hoxidase protein ratio in obese men. J Physiol 594: 2307–2321, 2016. doi:10.1113/jphysiol.2014. 285254. 17. Cocks M, Shaw CS, Shepherd SO, Fisher JP, Ranasinghe AM, Barker TA, Tipton KD, Wagenmakers AJ. Sprint interval and endurance training are equally effective in increasing muscle microvascular density and eNOS content in sedentary males. J Physiol 591: 641–656, 2013. doi:10.1113/jphysiol.2012.239566. 18. Cui G, Park S, Badeaux AI, Kim D, Lee J, Thompson JR, Yan F, Kaneko S, Yuan Z, Botuyan MV, Bedford MT, Cheng JQ, Mer G. PHF20 is an effector protein of p53 double lysine methylation that stabilizes and activates p53. Nat Struct Mol Biol 19: 916 –924, 2012. doi:10.1038/nsmb.2353. 19. Curran-Everett D. Multiple comparisons: philosophies and illustrations. Am J Physiol Regul Integr Comp Physiol 279: R1–R8, 2000. 20. Dresios J, Aschrafi A, Owens GC, Vanderklish PW, Edelman GM, Mauro VP. Cold stress-induced protein Rbm3 binds 60S ribosomal subunits, alters microRNA levels, and enhances global protein synthesis. Proc Natl Acad Sci USA 102: 1865–1870, 2005. doi:10.1073/pnas. 0409764102. 21. Evans WJ, Phinney SD, Young VR. Suction applied to a muscle biopsy maximizes sample size. Med Sci Sports Exerc 14: 101–102, 1982. 22. Gibala MJ, Little JP, van Essen M, Wilkin GP, Burgomaster KA, Safdar A, Raha S, Tarnopolsky MA. Short-term sprint interval versus traditional endurance training: similar initial adaptations in human skeletal muscle and exercise performance. J Physiol 575: 901–911, 2006. doi:10. 1113/jphysiol.2006.112094. 23. Gibala MJ, McGee SL. Metabolic adaptations to short-term high-intensity interval training: a little pain for a lot of gain? Exerc Sport Sci Rev 36: 58 –63, 2008. doi:10.1097/JES.0b013e318168ec1f. 24. Gibala MJ, McGee SL, Garnham AP, Howlett KF, Snow RJ, Hargreaves M. Brief intense interval exercise activates AMPK and p38 MAPK signaling and increases the expression of PGC-1 in human skeletal muscle. J Appl Physiol (1985) 106: 929 –934, 2009. doi:10.1152/ japplphysiol.90880.2008. 25. Gillen JB, Martin BJ, MacInnis MJ, Skelly LE, Tarnopolsky MA, Gibala MJ. Twelve weeks of sprint interval training improves indices of cardiometabolic health similar to traditional endurance training despite a five-fold lower exercise volume and time commitment. PLoS One 11: e0154075, 2016. doi:10.1371/journal.pone.0154075. 26. Gon Y, Hashimoto S, Matsumoto K, Nakayama T, Takeshita I, Horie T. Cooling and rewarming-induced IL-8 expression in human bronchial epithelial cells through p38 MAP kinase-dependent pathway. Biochem Biophys Res Commun 249: 156 –160, 1998. doi:10.1006/bbrc.1998.9115. 27. Granata C, Oliveira RS, Little JP, Renner K, Bishop DJ. Mitochondrial adaptations to high-volume exercise training are rapidly reversed after a reduction in training volume in human skeletal muscle. FASEB J 30: 3413–3423, 2016. doi:10.1096/fj.201500100R. 28. Granata C, Oliveira RS, Little JP, Renner K, Bishop DJ. Training intensity modulates changes in PGC-1 and p53 protein content and mitochondrial respiration, but not markers of mitochondrial content in human skeletal muscle. FASEB J 30: 959 –970, 2016. doi:10.1096/fj.15- 276907. 29. Halson SL, Bartram J, West N, Stephens J, Argus CK, Driller MW, Sargent C, Lastella M, Hopkins WG, Martin DT. Does hydrotherapy help or hinder adaptation to training in competitive cyclists? Med Sci Sports Exerc 46: 1631–1639, 2014. doi:10.1249/MSS. 0000000000000268. 30. Hawley JA, Noakes TD. Peak power output predicts maximal oxygen uptake and performance time in trained cyclists. Eur J Appl Physiol Occup Physiol 65: 79 –83, 1992. doi:10.1007/BF01466278. 31. Hellemans J, Mortier G, De Paepe A, Speleman F, Vandesompele J. qBase relative quantification framework and software for management and automated analysis of real-time quantitative PCR data. Genome Biol 8: R19, 2007. doi:10.1186/gb-2007-8-2-r19. 32. Higgins TR, Heazlewood IT, Climstein M. A random control trial of contrast baths and ice baths for recovery during competition in U/20 rugby union. J Strength Cond Res 25: 1046 –1051, 2011. doi:10.1519/JSC. 0b013e3181cc269f. 33. Ihsan M, Markworth JF, Watson G, Choo HC, Govus A, Pham T, Hickey A, Cameron-Smith D, Abbiss CR. Regular postexercise cooling enhances mitochondrial biogenesis through AMPK and p38 MAPK in human skeletal muscle. Am J Physiol Regul Integr Comp Physiol 309: R286 –R294, 2015. doi:10.1152/ajpregu.00031.2015. 34. Ihsan M, Watson G, Choo HC, Lewandowski P, Papazzo A, CameronSmith D, Abbiss CR. Postexercise muscle cooling enhances gene expression of PGC-1. Med Sci Sports Exerc 46: 1900 –1907, 2014. doi:10.1249/ MSS.0000000000000308. 35. Jäger S, Handschin C, St-Pierre J, Spiegelman BM. AMP-activated protein kinase (AMPK) action in skeletal muscle via direct phosphorylation of PGC-1alpha. Proc Natl Acad Sci USA 104: 12017–12022, 2007. doi:10.1073/pnas.0705070104. 36. Jiang HK, Wang YH, Sun L, He X, Zhao M, Feng ZH, Yu XJ, Zang WJ. Aerobic interval training attenuates mitochondrial dysfunction in rats post-myocardial infarction: roles of mitochondrial network dynamics. Int J Mol Sci 15: 5304 –5322, 2014. doi:10.3390/ijms15045304. 37. Joo CH, Allan R, Drust B, Close GL, Jeong TS, Bartlett JD, Mawhinney C, Louhelainen J, Morton JP, Gregson W. Passive and postexercise cold-water immersion augments PGC-1 and VEGF expression in human skeletal muscle. Eur J Appl Physiol 116: 2315–2326, 2016. doi:10.1007/s00421-016-3480-1. 38. Larsen S, Nielsen J, Hansen CN, Nielsen LB, Wibrand F, Stride N, Schroder HD, Boushel R, Helge JW, Dela F, Hey-Mogensen M. Biomarkers of mitochondrial content in skeletal muscle of healthy young human subjects. J Physiol 590: 3349 –3360, 2012. doi:10.1113/jphysiol. 2012.230185. 39. Li J, Donath S, Li Y, Qin D, Prabhakar BS, Li P. miR-30 regulates mitochondrial fission through targeting p53 and the dynamin-related protein-1 pathway. PLoS Genet 6: e1000795, 2010. doi:10.1371/journal. pgen.1000795. 40. Little JP, Safdar A, Bishop D, Tarnopolsky MA, Gibala MJ. An acute bout of high-intensity interval training increases the nuclear abundance of PGC-1 and activates mitochondrial biogenesis in human skeletal muscle. Am J Physiol Regul Integr Comp Physiol 300: R1303–R1310, 2011. doi:10.1152/ajpregu.00538.2010. 41. Ljubicic V, Joseph AM, Saleem A, Uguccioni G, Collu-Marchese M, Lai RY, Nguyen LM, Hood DA. Transcriptional and post-transcriptional regulation of mitochondrial biogenesis in skeletal muscle: effects of exercise and aging. Biochim Biophys Acta 1800: 223–234, 2010. doi:10. 1016/j.bbagen.2009.07.031. 42. Marzetti E, Groban L, Wohlgemuth SE, Lees HA, Lin M, Jobe H, Giovannini S, Leeuwenburgh C, Carter CS. Effects of short-term GH supplementation and treadmill exercise training on physical performance and skeletal muscle apoptosis in old rats. Am J Physiol Regul Integr Comp Physiol 294: R558 –R567, 2008. doi:10.1152/ajpregu.00620.2007. 43. Matijasevic Z, Snyder JE, Ludlum DB. Hypothermia causes a reversible, p53-mediated cell cycle arrest in cultured fibroblasts. Oncol Res 10: 605–610, 1998. 44. Matoba S, Kang JG, Patino WD, Wragg A, Boehm M, Gavrilova O, Hurley PJ, Bunz F, Hwang PM. p53 regulates mitochondrial respiration. Science 312: 1650 –1653, 2006. doi:10.1126/science.1126863. 45. Matsuo T, Saotome K, Seino S, Shimojo N, Matsushita A, Iemitsu M, Ohshima H, Tanaka K, Mukai C. Effects of a low-volume aerobic-type interval exercise on V˙ O2max and cardiac mass. Med Sci Sports Exerc 46: 42–50, 2014. doi:10.1249/MSS.0b013e3182a38da8. 46. Murphy RM, Lamb GD. Important considerations for protein analyses using antibody based techniques: down-sizing Western blotting up-sizes outcomes. J Physiol 591: 5823–5831, 2013. doi:10.1113/jphysiol.2013. 263251. 47. Newell J, Higgins D, Madden N, Cruickshank J, Einbeck J, McMillan K, McDonald R. Software for calculating blood lactate endurance markers. J Sports Sci 25: 1403–1409, 2007. doi:10.1080/02640410601128922. 48. Nishiyama H, Itoh K, Kaneko Y, Kishishita M, Yoshida O, Fujita J. A glycine-rich RNA-binding protein mediating cold-inducible suppression of mammalian cell growth. J Cell Biol 137: 899 –908, 1997. doi:10.1083/ jcb.137.4.899. 49. Oliveira RL, Ueno M, de Souza CT, Pereira-da-Silva M, Gasparetti AL, Bezzera RM, Alberici LC, Vercesi AE, Saad MJ, Velloso LA. Cold-induced PGC-1 expression modulates muscle glucose uptake through an insulin receptor/Akt-independent, AMPK-dependent pathway. Am J Physiol Endocrinol Metab 287: E686 –E695, 2004. doi:10.1152/ ajpendo.00103.2004. 50. Park JY, Wang PY, Matsumoto T, Sung HJ, Ma W, Choi JW, Anderson SA, Leary SC, Balaban RS, Kang JG, Hwang PM. p53 improves aerobic exercise capacity and augments skeletal muscle mitochondrial DNA content. Circ Res 105: 705–712, 2009. doi:10.1161/ CIRCRESAHA.109.205310. 51. Perry CG, Lally J, Holloway GP, Heigenhauser GJ, Bonen A, Spriet LL. Repeated transient mRNA bursts precede increases in transcriptional and mitochondrial proteins during training in human skeletal muscle. J Physiol 588: 4795–4810, 2010. doi:10.1113/jphysiol.2010.199448. 52. Pesta D, Gnaiger E. High-resolution respirometry: OXPHOS protocols for human cells and permeabilized fibers from small biopsies of human muscle. Methods Mol Biol 810, 25–58, 2012. doi:10.1007/978-1-61779-382-0_3. 53. Puigserver P, Wu Z, Park CW, Graves R, Wright M, Spiegelman BM. A cold-inducible coactivator of nuclear receptors linked to adaptive thermogenesis. Cell 92: 829 –839, 1998. doi:10.1016/S0092- 8674(00)81410-5. 54. Riley CL, Dao C, Kenaston MA, Muto L, Kohno S, Nowinski SM, Solmonson AD, Pfeiffer M, Sack MN, Lu Z, Fiermonte G, Sprague JE, Mills EM. The complementary and divergent roles of uncoupling proteins 1 and 3 in thermoregulation. J Physiol 594: 7455–7464, 2016. doi:10. 1113/JP272971. 55. Rossiter HB, Kowalchuk JM, Whipp BJ. A test to establish maximum O2 uptake despite no plateau in the O2 uptake response to ramp incremental exercise. J Appl Physiol (1985) 100: 764 –770, 2006. doi:10.1152/ japplphysiol.00932.2005. 56. Saleem A, Adhihetty PJ, Hood DA. Role of p53 in mitochondrial biogenesis and apoptosis in skeletal muscle. Physiol Genomics 37: 58 –66, 2009. doi:10.1152/physiolgenomics.90346.2008. 57. Saleem A, Carter HN, Hood DA. p53 is necessary for the adaptive changes in cellular milieu subsequent to an acute bout of endurance exercise. Am J Physiol Cell Physiol 306: C241–C249, 2014. doi:10.1152/ ajpcell.00270.2013. 58. Saleem A, Carter HN, Iqbal S, Hood DA. Role of p53 within the regulatory network controlling muscle mitochondrial biogenesis. Exerc Sport Sci Rev 39: 199 –205, 2011. doi:10.1097/JES.0b013e31822d71be. 59. Saleem A, Hood DA. Acute exercise induces tumour suppressor protein p53 translocation to the mitochondria and promotes a p53-Tfam-mitochondrial DNA complex in skeletal muscle. J Physiol 591: 3625–3636, 2013. doi:10.1113/jphysiol.2013.252791. 60. Schrauwen P. Skeletal muscle uncoupling protein 3 (UCP3): mitochondrial uncoupling protein in search of a function. Curr Opin Clin Nutr Metab Care 5: 265–270, 2002. doi:10.1097/00075197-200205000-00005. 61. Slivka D, Heesch M, Dumke C, Cuddy J, Hailes W, Ruby B. Effects of post-exercise recovery in a cold environment on muscle glycogen, PGC1, and downstream transcription factors. Cryobiology 66: 250 –255, 2013. doi:10.1016/j.cryobiol.2013.02.005. 62. Slivka DR, Dumke CL, Tucker TJ, Cuddy JS, Ruby B. Human mRNA response to exercise and temperature. Int J Sports Med 33: 94 –100, 2012. doi:10.1055/s-0031-1287799. 63. Sonna LA, Fujita J, Gaffin SL, Lilly CM. Invited review: effects of heat and cold stress on mammalian gene expression. J Appl Physiol (1985) 92: 1725–1742, 2002. doi:10.1152/japplphysiol.01143.2001. 64. Stambolsky P, Weisz L, Shats I, Klein Y, Goldfinger N, Oren M, Rotter V. Regulation of AIF expression by p53. Cell Death Differ 13: 2140 –2149, 2006. doi:10.1038/sj.cdd.4401965. 65. Tonkonogi M, Sahlin K. Rate of oxidative phosphorylation in isolated mitochondria from human skeletal muscle: effect of training status. Acta Physiol Scand 161: 345–353, 1997. doi:10.1046/j.1365-201X.1997. 00222.x. 66. Trilk JL, Singhal A, Bigelman KA, Cureton KJ. Effect of sprint interval training on circulatory function during exercise in sedentary, overweight/ obese women. Eur J Appl Physiol 111: 1591–1597, 2011. doi:10.1007/ s00421-010-1777-z. 67. Vaile J, Halson S, Gill N, Dawson B. Effect of hydrotherapy on recovery from fatigue. Int J Sports Med 29: 539 –544, 2008. doi:10.1055/s-2007- 989267. 68. Wang W, Cheng X, Lu J, Wei J, Fu G, Zhu F, Jia C, Zhou L, Xie H, Zheng S. Mitofusin-2 is a novel direct target of p53. Biochem Biophys Res Commun 400: 587–592, 2010. doi:10.1016/j.bbrc.2010.08.108. 69. Welinder C, Ekblad L. Coomassie staining as loading control in Western blot analysis. J Proteome Res 10: 1416 –1419, 2011. doi:10.1021/ pr1011476. 70. Wijers SL, Saris WH, van Marken Lichtenbelt WD. Recent advances in adaptive thermogenesis: potential implications for the treatment of obesity. Obesity Rev 10: 218 –226, 2009. doi:10.1111/j.1467-789X.2008. 00538.x. 71. Wilcock IM, Cronin JB, Hing WA. Physiological response to water immersion: a method for sport recovery? Sports Med 36: 747–765, 2006. doi:10.2165/00007256-200636090-00003. 72. Wu Z, Puigserver P, Andersson U, Zhang C, Adelmant G, Mootha V, Troy A, Cinti S, Lowell B, Scarpulla RC, Spiegelman BM. Mechanisms controlling mitochondrial biogenesis and respiration through the thermogenic coactivator PGC-1. Cell 98: 115–124, 1999. doi:10.1016/S0092- 8674(00)80611-X. 73. Yamanaka K. Cold shock response in Escherichia coli. J Mol Microbiol Biotechnol 1: 193–202, 1999. 74. Yamane M, Teruya H, Nakano M, Ogai R, Ohnishi N, Kosaka M. Post-exercise leg and forearm flexor muscle cooling in humans attenuates endurance and resistance training effects on muscle performance and on circulatory adaptation. Eur J Appl Physiol 96: 572–580, 2006. doi:10. 1007/s00421-005-0095-3.